A coronavirus uses a protein on its membrane—shown here in red in a molecular model—to bind to a receptor—shown in blue—on a human cell to enter the cell. Once inside, the virus uses the cells' machinery to make more copies of itself. (Juan Gaertner / Science Source)
Rochester research into RNA structure and function provides key information for developing coronavirus treatments.
Viruses like the coronavirus that causes COVID-19 are able to unleash their fury because of a devious weapon: ribonucleic acid, also known as RNA.
A contingent of researchers at the University of Rochester study the RNA of viruses to better understand how RNAs work and how they are involved in diseases. As COVID-19 continues to spread around the globe, this research provides an important foundation for developing antiviral drugs, vaccines, and other therapeutics to disrupt the virus and stop infections.
Coronavirus update
The University’s website is a way to find guidance and critical information during a rapidly changing situation.
COVID-19 symptoms or exposure?
Find out what to do if you or a close contact have symptoms or think you may have been exposed.
“Understanding RNA structure and function helps us understand how to throw a therapeutic wrench into what the COVID-19 RNA does—make new virus that can infect more of our cells and also the cells of other human beings,” says Lynne Maquat, professor of biochemistry and biophysics at the University of Rochester Medical Center and the director of Rochester’s Center for RNA Biology.
In the past few decades, as scientists came to realize that genetic material is largely regulated by the RNA it encodes, that most of our DNA produces RNA, and that RNA is not only a target but also a tool for disease therapies, “the RNA research world has exploded,” Maquat says. “The University of Rochester understood this.”
In 2007, Maquat founded the Center for RNA Biology as a means of conducting interdisciplinary research in the function, structure, and processing of RNAs. The center involves researchers from both the River Campus and the Medical Center, combining expertise in biology, chemistry, engineering, neurology, and pharmacology.
While much of the research across the University has been put on pause, labs that are involved in coronavirus research remain active.
“Our strength as a university is our diversity of research expertise, combined with our highly collaborative nature,” says Dragony Fu, an assistant professor of biology on the River Campus and a member of the Center for RNA Biology. “We are surrounded by outstanding researchers who enhance our understanding of RNA biology, and a medical center that provides a translational aspect where the knowledge gained from RNA biology can be applied for therapeutics.”
How does coronavirus infect humans?
In mammals, such as humans, DNA contains genetic instructions that are transcribed—or copied—into RNA. While DNA remains in the cell’s nucleus, RNA carries the copies of genetic information to the rest of the cell by way of various combinations of amino acids, which it delivers to ribosomes. The ribosomes link the amino acids together to form proteins that then carry out functions within the human body.
Many diseases occur when these gene expressions go awry.
What does RNA stand for?
RNA stands for ribonucleic acid.
What is RNA?
RNA delivers the genetic instructions contained in DNA to the rest of the cell.
COVID-19, short for “coronavirus disease 2019,” is caused by the novel coronavirus SARS-CoV-2. Like many other viruses, SARS-CoV-2 is an RNA virus. This means that, unlike in humans and other mammals, the genetic material for SARS-CoV-2 is encoded in ribonucleic acid. The viral RNA is sneaky: its features cause the protein synthesis machinery of our cells to mistake it for RNA produced by our own DNA.
While SARS-CoV-2 is a new coronavirus, “it likely replicates and functions similar to related coronaviruses that infect animals and humans,” says Douglas Anderson, an assistant professor of medicine in the Aab Cardiovascular Research Institute and a member of the Center for RNA Biology, who studies how RNA mutations can give rise to human disease.
A graphic created by the New York Times illustrates how the coronavirus that causes COVID-19 enters the body through the nose, mouth, or eyes and attaches to our cells. Once the virus is inside our cells, it releases its ribonucleic acid. Our hijacked cells serve as virus factories, reading the virus’s ribonucleic acid and making long viral proteins to compromise the immune system. The virus assembles new copies of itself and spreads to more parts of the body and—by way of saliva, sweat, and other bodily fluids—to other humans.
“Once the virus is in our cells, the entire process of infection and re-infection depends on the viral RNA,” Maquat says.
How is Rochester’s RNA research applicable to COVID-19?
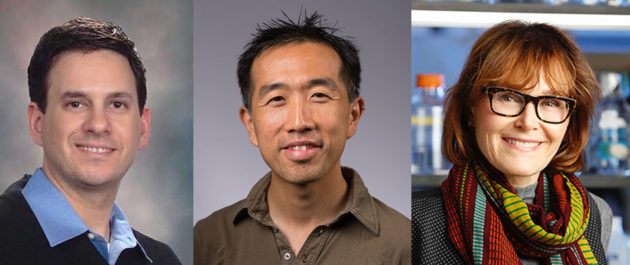
Researchers Douglas Anderson, Dragony Fu, and Lynne Maquat are among the scientists at the University of Rochester who study the RNA of viruses to better understand how RNAs work and how they are involved in diseases. (University of Rochester photos / Matt Wittmeyer / J. Adam Fenster)
Maquat has been studying RNA since 1972 and was part of the earliest wave of scientists to realize the important role RNA plays in human health and disease.
Our cells have a number of ways to combat viruses in what can be viewed as an “arms race” between host and virus. One of the weapons in our cells’ arsenal is an RNA surveillance mechanism Maquat discovered called nonsense-mediated mRNA decay (NMD).
“Nonsense-mediated mRNA decay protects us from many genetic mutations that could cause disease if NMD was not active to destroy the RNA harboring the mutation,” she says.
Maquat’s discovery has contributed to the development of drug therapies for genetic disorders such as cystic fibrosis, and may be useful in developing treatments for coronavirus.
“NMD also helps us combat viral infections, which is why many viruses either inhibit or evade NMD,” she adds. “The genome of the virus COVID-19 is a positive-sense, single-stranded RNA. It is well known that other positive-sense, single-stranded RNA viruses evade NMD by having RNA structures that prevent NMD from degrading viral RNAs.”
Maquat’s lab is currently collaborating with a lab at Harvard University to test how viral proteins can inhibit the NMD machinery.
Like Maquat, Fu studies fundamental aspects of RNA—and has found that his research on proteins may, too, be applicable to coronavirus research.
Fu’s lab analyzes enzymes and proteins that modify the chemical structure of RNA and how these chemical modifications impact the function of RNA. A research group at the University of California, San Francisco, recently identified an interaction between a protein made by the SARS-CoV-9 virus and a protein Fu studies.
“This is an intriguing result, and we are currently thinking of ways this interaction could affect the host cell,” Fu says. “There is emerging evidence that RNA-based viruses undergo RNA modification, so we could use this knowledge to identify key links between the host and pathogen for development of a coronavirus vaccine or treatment.”
How can RNA research be used to develop coronavirus treatments and vaccines?
One of the reasons viruses are such a challenge is that they change and mutate in response to drugs.
“Targeting viral RNA, or the proteins it produces, is key for treating this disease.”
That means novel virus treatments and vaccines have to be created each time a new strain of virus presents itself. Armed with innovative research on the fundamentals of RNA, scientists are better able to develop and test therapeutics that directly target the RNAs and processes critical to a virus’s life cycle.
The University of Rochester Medical Center, for instance, is currently participating in a clinical trial to evaluate the safety and efficacy of a potential coronavirus treatment called remdesivir, an antiviral drug particularly tailored to attack RNA viruses. The drug inhibits RNA polymerase, an enzyme responsible for copying a DNA sequence into an RNA sequence.
The Medical Center is additionally collaborating on a clinical trial to test the efficacy of an RNA vaccine. Traditional vaccines against viruses like influenza inject the body with inactivated virus proteins called antigens. The antigens stimulate the body’s immune system to recognize the specific virus and produce antibodies in response, with the hope that these antibodies will fight against future virus infection.
RNA vaccines, on the other hand, do not introduce an antigen, but instead inject a short sequence of the virus’s RNA. This messenger RNA provides cells with instructions to produce the virus antigens themselves. While no RNA vaccines have yet been approved in advanced vaccine trials, these vaccines have an advantage over traditional vaccines in that they would be easier and quicker to produce, and would allow the body to generate a more natural response to a virus.
Anderson has found that alternative therapeutics, such as the gene-editing technology CRISPR, may additionally “usher in a new approach to how we target and combat infectious diseases,” he says.
For the past few years, Anderson’s lab has developed tools and delivery systems that use the RNA-targeting CRISPR-Cas13 to treat human genetic diseases that affect muscle function. CRISPR-Cas13 is like a molecular pair of scissors that can target specific RNAs for degradation, using small, programmable guide RNAs.
When the health crisis first became apparent in Wuhan, China, researchers in Anderson’s lab turned their focus toward developing a CRISPR-Cas13 therapeutic aimed at SARS-CoV-2. Applying the knowledge already available about coronavirus RNA replication, they designed single CRISPR guide RNAs capable of targeting every viral RNA that is made within a SARS-CoV-2 infected cell. Using a novel cloning method developed in Anderson’s lab, multiple CRISPR guide-RNAs could be packaged into a single therapeutic vector (a genetically engineered carrier) to target numerous viral RNA sites simultaneously. The multi-pronged targeting strategy could be used as a therapy to safeguard against virus-induced cell toxicity and prevent ‘escape’ of viruses which may have undergone mutation.
“Infectious viruses and pandemics seemingly come out of nowhere, which has made it hard to rapidly develop and screen traditional small molecule therapeutics or vaccines,” Anderson says. “There is a clear need to develop alternative targeted therapeutics, such as CRISPR-Cas13, which have the ability to be rapidly reprogrammed to target new emerging pandemics.”
While many new treatments for the novel coronavirus are being considered, there is one thing that is certain, Maquat says: “Targeting viral RNA, or the proteins it produces, is key for treating this disease.”
Read more
New URMC coronavirus research examines immune responseResearchers at the New York Influenza Center of Excellence are launching a new study to determine if and when a person could be re-infected with the novel coronavirus and whether some people have pre-existing immunity.
Tags: Arts and Sciences, Center for RNA Biology, COVID-19, Department of Biochemistry and Biophysics, Department of Biology, Douglas Anderson, Dragony Fu, featured-post, Lynne Maquat, medical center
Category: Science & Technology